Aerogels as porous structures for food applications: Smart ingredients and novel packaging materials
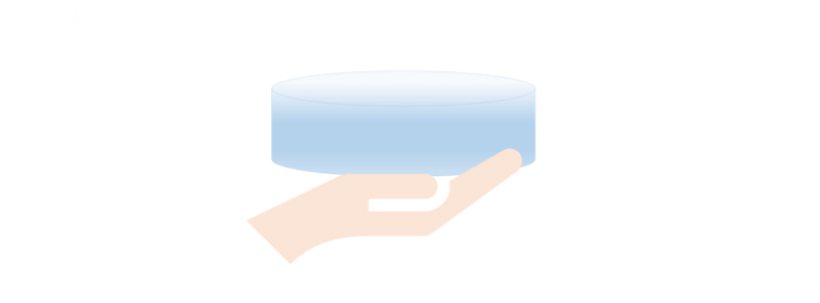
LaraManzoccoaKirsi S.MikkonenbcCarlos A.García-Gonzálezd
aDipartimento di Scienze AgroAlimentari, Ambientali e Animali, Università di Udine, Via Sondrio 2/A, Udine, I-33100, Italy bDepartment of Food and Nutrition, P.O. Box 66 (Agnes Sjöbergin katu 2), University of Helsinki, FI-00014, Finland cHelsinki Institute of Sustainability Science, Faculty of Agriculture and Forestry, University of Helsinki, FI-00014, Finland dDept. Farmacología, Farmacia y Tecnología Farmacéutica, I+D Farma Group (GI-1645), Faculty of Pharmacy, and Health Research Institute of Santiago de Compostela (IDIS), Universidade de Santiago de Compostela, Santiago de Compostela, 15782, Spain
https://doi.org/10.1016/j.foostr.2021.100188 Received 19 December 2020, Revised 17 February 2021, Accepted 19 February 2021, Available online 23 February 2021. 2213-3291/© 2021 The Author(s). Published by Elsevier Ltd. This is an open access article under the CC BY license (http://creativecommons.org/licenses/by/4.0/). |
Abstract
Aerogels are nanostructured materials with low density, high surface area (>150 m2/g) and open porosity (typically 95–99.99 %). They are obtained by solvent removal from gels while preserving network structure. Hydrogels, organogels and even tissues can be optimal sources of aerogels with limitless customization of format and texture. Aerogels might be used for a range of advanced food applications: from smart ingredients controlling nutrient release to delivery systems for active compounds; from fat substitutes to novel biodegradable and intelligent food packaging materials. This review article summarizes recent developments of aerogels in food applications, analyzing research challenges and prospecting future markets.
1. Introduction
The advent of nanostructured materials for food applications is rather recent and mainly focused on nanoencapsulation of ingredients (protection, masking, flavoring, tuned bioavailability), packaging and nanosensors (Ghanbarzadeh, Oleyaei, & Almasi, 2015; Pathakoti, Manubolu, & Hwang, 2017). The most common approaches used for these purposes are micro- and nanoemulsions as well as liposomes but there is still a broad room for food research on other nanostructured materials.
Aerogels are defined as a special type of nanostructured material endowed with special physical features and obtained from a gel by removing the pore fluid (García-González et al., 2019). Solid, low bulk density and open porosity (mainly in the mesoporous range) stand out as specific physical properties that the material should fulfill to fit in the consensual definition of aerogel. Aerogel networks are formed by bonded particles or nanometric fibers that are loosely packed leading to high porosities (typically in the 95–99.99 % range) and very high specific surface areas (150 m2/g and above) (Fig. 1). These structures result in unique thermal and sound insulation properties and high loading capacities that are being exploited in many industrial fields (aerospace, buildings, petrochemical) and are under research in the recent years for environmental and biomedical applications (www.cost-aerogels.eu), including functional food and packaging (Lehtonen et al., 2020; Plazzotta, Calligaris, & Manzocco, 2018; Plazzotta, Calligaris, & Manzocco, 2018; Plazzotta, Calligaris, & Manzocco, 2020; Selmer et al., 2019).
Fig. 1. SEM images of the microstructures of (a) alginate and (b) pectin aerogels obtained by supercritical drying, (c) cellulose aerogels by freeze-drying, and (d) silica-cellulose composite aerogels by ambient drying.
Adapted from https://doi.org/10.1016/j.cej.2018.09.159, https://doi.org/10.1016/j.carbpol.2018.05.026, https://doi.org/10.1021/acsami.5b05841, https://doi.org/10.1007/s10853-016-0514-3 with permissions. |
Aerogels are obtained by the extraction of the solvent of wet gels (hydrogels or organogels) using a method that allows the preservation of the solid network structure, typically supercritical carbon dioxide (scCO2)-assisted drying being the gold-standard (Fig. 1a,b). Fig. 2 shows a schematization of the possible strategies to turn hydrogels into aerogels. Prior to supercritical drying, a solvent exchange may be needed depending on the gel solvent, as the solubility of water in supercritical CO2 is low but the solubility of ethanol or acetone is high (Şahin, Özbakır, İnönü, Ulker, & Erkey, 2017). Other drying techniques, such as ambient/oven drying or freeze drying, can also lead to aerogel structures in very specific cases (Fig. 1c,d). For ambient/oven drying, surface functionalization (mainly with silanes) or flexible gels followed by the sequential use of solvents with low surface tension (hexane, pentane, ethanol, acetone) may be needed to avoid the shrinkage or cracking of the dry gel due to capillary forces (Budtova, 2019). For freeze drying of hydrogels, the higher volume of water in the solid than in the liquid form results in solvent expansion upon solidification with severe porous damages usually leading to the formation of foams with large macropores, microchannels, cracks and loss of mesoporosity after sublimation (Fig. 3b) (Baudron, Gurikov, Smirnova, & Whitehouse, 2019; Rodríguez-Dorado et al., 2019). Despite these limitations, certain biopolymer-based aerogels, typically nanofibrous cellulose aerogels but also chitin aerogels, may be also obtained by atmospheric drying or freeze-drying (Budtova, 2019; Gao, Lu, Xiao, & Li, 2017; Jiménez-Saelices, Seantier, Cathala, & Grohens, 2017; Li et al., 2019; Nemoto, Saito, & Isogai, 2015).
Fig. 2. Flow sheet (continuous line) and addition of materials (dashed line) to obtain aerogels and functionalized aerogels. Steps at which target molecule is impregnated or oil is absorbed are also shown.
Fig. 3. Aerogel engineering strategies: (a) Shape preservation; visual appearance of starch, and pectin hydrogels and their corresponding aerogels. (b) Effect of drying technique; optical appearance of a whey protein hydrogel prepared at pH 7 and the corresponding alcogels, aerogels and cryogels (above). The microstructure of the whey protein aerogels (bottom left) and cryogels (bottom right) obtained by supercritical drying and freeze-drying differed significantly in morphology and textural properties (Sa=specific surface area determined from N2 adsorption-desorption analysis). (c) Customized morphology; aerogels monoliths with personalized shape, aerogel particles from the millimetric to the micrometric sizes and coated aerogels.
Adapted from https://doi.org/10.1016/j.supflu.2013.03.001, https://doi.org/10.1016/j.carbpol.2011.06.066, https://doi.org/10.1016/j.supflu.2012.08.019, https://doi.org/10.1038/s41586-020-2594-0, https://doi.org/10.1016/j.cej.2018.09.159, https://doi.org/10.1016/j.powtec.2015. |
A limitless customization of the format, shape, size and texture of the aerogels is possible and is mainly carried out during the gelation step using different molds and gelation conditions (gel source concentration, cross-linker concentration, etc.) (Fig. 1, Fig. 3) (García-Gonzalez, Alnaief, & Smirnova, 2011). For the specific case of aerogel particles, the use of molds is usually replaced by technological combinations of the sol-gel processing with powder technologies (e.g., emulsion-gelation, spraying, prilling, inkjet printing, jet-cutting) or by postprocessing (grinding) (Auriemma et al., 2020; Ganesan et al., 2018). The size and texture of the aerogels can be also partially modulated during the solvent exchange step (solvent choice, direct or sequential procedure) or by post-processing (aerogel compression) (García-Gonzalez et al., 2011; Plappert, Nedelec, Rennhofer, Lichtenegger, & Liebner, 2017). Finally, aerogels with dual formats like core-shell aerogel particles and coated aerogel particles are also possible by technological combinations (Antonyuk, Heinrich, Gurikov, Subrahmanyam, & Smirnova, 2015; Auriemma et al., 2020; Bugnone, Ronchetti, Manna, & Banchero., 2018; Veronovski, Knez, & Novak, 2013).
Most aerogels are inorganic or synthetic polymer-based, being often made of silica, metal oxides or polystyrenes (Du, Zhou, Zhang, & Shen, 2013; Gesser & Goswami, 1989). However, according to different authors (Kistler, 1931; Pierre & Pajonk, 2002, 1932; Zhao, Malfait, Guerrero‐Alburquerque, Koebel, & Nyström, 2018), not only inorganic polymerizing agents but all biopolymers are potential candidates to form aerogels. Namely, the second generation of aerogels eases the penetration of these materials in the food market since it comprises biopolymer-based aerogels, including food-grade polysaccharides and proteins (El-Naggar, Othman, Allam, & Morsy, 2020; García-Gonzalez et al., 2011; Nita, Ghilan, Rusu, Neamtu, & Chiriac, 2020). These new aerogel sources provided new opportunities for food applications due to their compatibility with human diet, absence of adverse health effects and peculiar physical properties. Aerogels can be used as edible delivery systems for nutraceuticals, nutritional supplements, flavors and other additives or as intelligent components for food packaging. Aerogels can be designed to serve as hosts or carriers of food ingredients and can increase the stability of the loaded ingredient, mask its odor and allow for a controlled or pH-triggered release after intake (Betz, García-Gonzalez, Subrahmanyam, Smirnova, & Koluzik, 2012; Del Gaudio et al., 2013; García-González, Jin, Gerth, Alvarez-Lorenzo, & Smirnova, 2015; Tkalec, Knez, & Novak, 2016). Food-grade aerogels can also contribute to an increased shelf life of the product by the encapsulation of labile or sensitive components (De Oliveira et al., 2020; García-González et al., 2021; Miranda-Tavares, Croguennec, Carvalho, & Bouhallab, 2014). The use of natural polymers can be also considered as an economical and environmentally-friendly approach to penetrate the food packaging sector.
Finally, aerogels as nanostructured materials should be assessed regarding food safety, mainly with respect to the overproduction of reactive oxygen species inducing cell oxidative stresses (Eleftheriadou, Pyrgiotakis, & Demokritou, 2017; Fu, Xia, Hwang, Ray, & Yu, 2014; Pathakoti et al., 2017). Accordingly, the changes in physicochemical and biological properties of aerogels with respect to the bulk unprocessed counterpart should be critically studied in food systems.
In this review article, the current state-of-the-art of aerogel processing for food applications is compiled for the first time. This work will firstly focus on different biopolymer-based aerogel sources obtained from hydrogels, organogels and tissues as the main sources of food-grade aerogels. Then, the evaluation of these aerogels for their potential direct uses as functional ingredients by themselves, as delivery systems and as fat replacers in food products, as well as their indirect food application in packaging are discussed. Finally, current gaps and challenges in aerogel research for food are identified, and future niche markets for food-grade aerogels are prospected.
2. Bio-based materials for aerogel preparation
The preparation of aerogels intended for food applications is virtually possible from any bio-based material characterized by a tridimensional polymeric network. Table 1 compares the number of papers published on aerogels during the last two decades, in relation to specific materials that might be used for their preparation. The research was performed using not only Web of Science platform but also Food Science and Technology Abstracts ones to highlight current interest of food scientists towards aerogel materials. Data clearly unveiled that biomedical and environmental applications of aerogels are two important directions of current mainstream research. By contrast, research on aerogels intended for food applications is in its early steps and their full potential is still to be assessed. Following, the main bio-based materials that can be used for preparation of aerogels for food applications are discussed.
Table 1. Number of articles on bio-based aerogels indexed in the Web of Science and the Food Science and Technology Abstracts platforms.
Material | Building blocks | Biopolymer | Number of papers | |
---|---|---|---|---|
Web of Science | Food Science and Technology Abstracts | |||
Hydrogel | Carbohydrates | Cellulose | 1129 | 13 |
Hemicellulose | ||||
-Glucomannan |
28 | 2 | ||
-β-glucan |
10 | 2 | ||
-Xylan |
4 | – | ||
-Xyloglucan |
4 | – | ||
Alginate | 231 | 2 | ||
Chitin | 65 | – | ||
Starch | 91 | 6 | ||
Pectin | 33 | – | ||
Carrageenan | 23 | 1 | ||
Garose | 24 | 1 | ||
Gums | ||||
-Gellan gum |
1 | – | ||
-Xanthan gum |
6 | 2 | ||
-Guar gum |
12 | – | ||
-Locust bean gum |
– | – | ||
Hyaluronan | – | – | ||
Aminoacids | Gelatin | 52 | 1 | |
Collagen | 24 | – | ||
Whey proteins | 8 | 4 | ||
Caseinate | 2 | 2 | ||
Egg white | 6 | 4 | ||
Phenols | Lignin | – | – | |
Nucleotides | Polynucleotides | – | – | |
Organogel | Carbohydrates | Ethylcellulose | – | 1 |
Chitin “wiskers” | – | – | ||
Aminoacids | Proteins | – | – | |
Tissues | 1 | 103 |
Search criteria: “aerogel” AND “name of biopolymer”; “aerogel” AND “tissue”. Search date: 28/11/2020.
2.1. Hydrogels
2.1.1. Polysaccharide hydrogels
At present, the majority of bio-based aerogels are obtained from polysaccharide hydrogels (Table 1). To this regard, the prospects of polysaccharide hydrogels for the production of aerogels have been discussed by different authors (Baudron, Taboada, Gurikov, Smirnova, & Whitehouse, 2020; García-Gonzalez et al., 2011; Zheng, Tian, Ye, Zhou, & Zhao, 2020). Nevertheless, applications relevant to the food sector are limited and mainly relevant to the use of cellulose and starch (Table 1) (Ivanovic, Milovanovic, & Zizovic, 2016; Mikkonen, Parikka, Ghafar, & Tenkanen, 2013; Ubeyitogullari & Ciftci, 2016). Beside them, other polysaccharides traditionally used as food thickeners or dietetic fiber, have the potential of networking, begetting gels which could be turned into aerogels. This possibility has been demonstrated with reference to hemicelluloses (Comin, Temelli, & Saldaña, 2012; Mikkonen et al., 2014; Parikka et al., 2017; Ubeyitogullari & Ciftci, 2020), pectin (White, Budarin, & Clark, 2010), alginates (Alnaief, Alzaitoun, García-Gonzalez, & Smirnova, 2011; Escudero, Robitzer, Di Renzo, & Quignard, 2009; Mallepally, Bernard, Marin, Ward, & McHugh, 2013), xanthan gum (Bilanovic, Starosvetsky, & Armon, 2016) and carrageenan (Manzocco et al., 2017).
2.1.2. Protein hydrogels
Gelatin and collagen are certainly the most studied proteins for aerogel preparation, being particularly suitable not only as drug carriers but also for the development of scaffolds for regenerative medicine and plastic surgery (Betz et al., 2012; Liu et al., 2019; Mehling, Smirnova, Guenther, & Neubert, 2009; Munoz-Ruiz et al., 2019; Zeynep & Erkey, 2014). By contrast, literature evidence about protein aerogels for food application is basically focus on dairy and egg white proteins (Chen, Wang, & Schiraldi, 2013; Kleemann, Selmer, Smirnova, & Kulozik, 2018; Selmer, Kleemann, Kulozik, Heinrich, & Smirnova, 2015). Proteins are also used in combination with other biopolymers to drive the internal morphology of composite aerogels. For instance, soy proteins have been demonstrated to be suitable for controlling the transition from fibrillar- to network-like architecture in composite protein-cellulose aerogels (Arboleda et al., 2013), while zein has been suggested as sacrificial porogen to obtain macropores within continuous starch aerogels (Santos-Rosales et al., 2019).
2.1.3. Hydrogels from other biopolymers
Recently, it has also been demonstrated that not only biopolymers with building blocks of saccharides or aminoacids can beget gels, but also those made of polyphenolic compounds, such as lignin, which might gel upon cross-linking (Li, Ge, & Wan, 2015). In addition, polynucleotides seem to be excellent components for the construction of hydrogels with tunable mechanical properties (Gačanin, Synatschke, & Weil, 2019). This is due to the fact that the gel network is stabilized by covalent bonding and not by low energy and non-specific interactions, as occurs in polysaccharides.
2.2. Organogels
Despite the high number of literature results on aerogel preparation from hydrogels, information about the possibility of obtaining aerogels from organogels is almost absent. An organogel can be defined as tridimensional networks entrapping an organic liquid (Co & Marangoni, 2012; Patel & Dewettinck, 2016; Térech & Weiss, 1997). In the food sector, this organic fluid is often represented by oil, which accounts for the use of the organogel synonym “oleogel”. Most oleogelators are low molecular weight compounds that self-assemble to form thermo-reversible organogels. The latter are likely to be unsuitable for aerogel production. However, more recently, it has been demonstrated that even large biopolymers could be used for organogelation. Examples of biopolymers able to network in oil are cellulose derivative ethylcellulose, hydrophobic chitin “whiskers” and protein emulsions/foams (Davidovich-Pinhas, Barbut, & Marangoni, 2015; Huang et al., 2015; Laredo, Barbut, & Marangoni, 2011; Nikiforidis & Scholten, 2015; Patel, 2018; Romoscanu & Mezzenga, 2006). Removal of the solvent from these oleogels could be applied to turn them into highly lipophilic aerogels, with unique oil absorption features. The feasibility of producing hydrophobic aerogels from oleogels was recently investigated, allowing scaffolds entrapping 0.6 g oil/g (Manzocco, Basso, Plazzotta, & Calligaris, 2021).
2.3. Tissues
It has been inferred that even tissues could represent optimal candidates for the preparation of bioaerogel-like materials (Plazzotta et al., 2018b). For instance, vegetable matrices can be regarded as complex networks of cellulose, embedding water within intra- and inter-cellular spaces. Adequate drying of fresh-cut salad waste actually allowed obtaining aerogel-like materials with high internal surface (>100 m2/g) and low density (<0.5 g/cm3) (Plazzotta et al., 2018a, 2018b). The use of tissues for aerogel preparation could present the advantage of simplifying the production process, since the gelling phase is not required. In addition, development of aerogel from vegetable or animal wastes could allow valorization of industrial discards, which typically represent an environmental and economic burden.
3. Applications of aerogels in food
Aerogels are porous materials, mainly occupied by air, which could find applications as low-calorie ingredients, able to tune nutrient release and modulate satiety. In addition, because of the large surface area and open pore structure, aerogels can accommodate several components, begetting a full range of functionalized derivatives. Fig. 2 shows a schematization of the possible strategies for aerogels functionalization. From one side, aerogels can be used to protect and deliver target molecules, potentially triggered by adverse environmental conditions or undesired tastes and odors. On the other hand, based on their capacity to entrap large amount of unsaturated lipids, aerogels could also be regarded as promising sources for the preparation of fat substitutes with health protecting capacity.
3.1. Functional ingredients
Aerogel production steps (e.g. gel formation, solvent exchange, drying) are expected to modify the physical structure and the chemical interactions among the biopolymers used for their preparation. It is thus likely that diffusion, erosion, swelling and fragmentation during digestion of polymer chains within an aerogel structure would occur according to rates other than those typically associated to the unstructured polymer. Similarly, environmental conditions affecting digestion would be significantly altered. This opens up brand new possibilities for using aerogels as promising functional ingredients. In fact, in the simplest case, a functional food is requested to tune the release of nutritional compounds in the gastrointestinal tract, according to the specific consumer needs. For instance, there is a growing interest in technological strategies to increase the amount of resistant starch in the diet. Resistant starch has actually the potential of improving human health by protecting against diseases such as colon cancer, type 2 diabetes and obesity. In this context, Ubeyitogullari, Brahma, Rose, and Ciftci (2018) have demonstrated that wheat starch aerogels obtained from starch gelatinised at 120 °C provided a 4.5-fold increase in resistant starch content, even after cooking. On the other hand, highly porous aerogel particles could also be regarded as empty fillers, being inert or active depending on the occurrence of interactions with the other food components. In addition, the proportion of air inclusion in a gel matrix is known to improve the food sensory properties by enhancing taste and flavour perception. This is attributed to a higher diffusion rate of tastants when air is included, and contributes to an increased delivery and perception of saltiness, sweetness and flavor (Chiu, Hewson, Yang, Linforth, & Fisk, 2015; Goh, Leroux, Groeneschild, & Busch, 2010). In this sense, the introduction of aerogels in foods would represent an additional strategy to reduce energy and salt intake through the diet (Osterholt, Liane, Roe, & Rolls, 2007). Nevertheless, studies on the compatibility of aerogels with other food components during food processing and storage are almost negligible, and no information is available on the effect of aerogels on food sensory properties and consumer acceptability.
3.2. Delivery systems
The possibility of using aerogels as novel carriers has shown great promise and is certainly the most studied application in the food sector. Basically, the loading of the target compound in the aerogel can be performed at any step of its preparation (Fig. 2). Depending on this choice, two main strategies of aerogel functionalisation can be identified: wet and post drying impregnation. Table 2 compares the efficacy of these techniques when applied to impregnate differently prepared aerogels.
Table 2. Main functionalisation strategies and amount of loaded compound in aerogels of different nature and shape, and prepared according to different drying techniques. Literature references are also reported.
Functionalisation strategy | Aerogel nature | Drying technique | Aerogel shape | Loaded compound | Loading (g/g aerogel) | Literature reference | |
---|---|---|---|---|---|---|---|
Wet impregnation | in water | Glucomannan | FD | M | Sunflower oil | <0.8 | Lehtonen et al. (2020) |
β-glucan | SCD | M | Flax oil | <0.1 | Comin et al. (2012) | ||
β-glucan | SCD | M | Flax lignan | <0.1 | Comin, Temelli, and Saldaña (2015) | ||
in ethanol | Bacterial cellulose | SCD | M | Vitamin C | 0.3 | Haimer et al. (2010) | |
Alginate | SCD | P | Resveratrol | 0.6 | Dos Santos et al. (2020) | ||
Alginate | SCD | P | Passion fruit extract | 0.6 | Viganó et al. (2020) | ||
in SC CO2 | β-glucan | SCD | M | Flax oil | 1.4 | Comin et al. (2012) | |
Post drying impregnation | without assisting solvent | Starch | FD | M | Trans-2- hexanal | n.r. | Abhari, Madadlou, and Dini (2017) |
hexane-assisted | Whey protein | FD | M | Fish oil | 2.6 | Ahmadi et al. (2016) | |
SC CO2-assisted | Whey protein | SCD | P | Fish oil | 0.7 | Kleemann et al. (2020) and Selmer et al. (2019) | |
Egg white protein | SCD | P | Fish oil | 0.7 | Kleemann et al. (2020) and Selmer et al. (2019) | ||
Sodium caseinate | SCD | P | Fish oil | 0.2 | Kleemann et al. (2020) and Selmer et al. (2019) | ||
Starch | SCD | M | α-tocopherol | 0.2 | De Marco & Reverchon, 2017 | ||
Starch | SCD | M | Vitamin K3 | <0.1 | De Marco and Reverchon (2017) | ||
Alginate | SCD | S | Vitamin D3 | <0.1 | Pantić, Knez, and Novak (2016) and Pantić, Kotnik, Knez, and Novak (2016) | ||
Alginate | SCD | P | Benzoic acid | 0.2 | García-González et al. (2015) | ||
Pectin | SCD | P | Benzoic acid | 0.1 | García-González et al. (2015) | ||
Starch | SCD | P | Benzoic acid | 0.2 | García-González et al. (2015) | ||
Starch | SCD | M | Phytosterols | 0.1 | Ubeyitogullari and Ciftci (2019) | ||
Chitosan | SCD | M | Lactulose | <0.1 | Díez-Municio et al. (2011) | ||
Chitosan | SCD | P | Lactulose | <0.1 | Díez-Municio et al. (2011) | ||
Post drying oil absorption | without assisting solvent | Iceberg salad | FD | M | Sunflower oil | 3.2 | Plazzotta et al. (2018b) |
Whey protein | FD | P | Sunflower oil | 2.3 | Plazzotta et al. (2020) | ||
k-carrageenan | SCD | M | Sunflower oil | 4.3 | Manzocco et al. (2017) | ||
Whey protein | SCD | P | Sunflower oil | 5.6 | Plazzotta et al. (2020) |
n.r. Not reported. M: Monoliths; P: Particles; S: Spheres; FD: freeze-drying; SCD: supercritical drying.
3.2.1. Wet impregnation
If target molecule incorporation is performed before drying, the process is generally referred to as wet impregnation (Fig. 2). In this case, the target compound is dissolved in one of the solvents that come into contact with the biopolymer during aerogel preparation (water or ethanol). Alternatively, the target molecule can be vehiculated by the SC-CO2 flow during supercritical drying of the alcogel following a drying impregnation approach (Comin et al., 2012). For instance, the target molecule could be simply inserted in the aqueous or ethanol solution used for hydrogel and alcogel preparation, respectively. This approach requires the molecule to exert a certain affinity to the selected solvent as well as to be resistant to environmental conditions of polymer gelation (e.g., high temperature, extreme pH and ionic force) and/or subsequent steps (solvent exchange and drying). Wet impregnation can be also performed into the alcogel. In this case, the alcogel is soaked in an ethanol solution containing the target molecule for a specific time. Following, drying is carried out using supercritical CO2, which extracts ethanol and causes precipitation of the target molecule within the aerogel pores by an antisolvent mechanism (Miguel, Martín, Gamse, & Cocero, 2006). The efficacy of aerogel wet impregnation is strongly dependent on the target molecule-solvent affinity (Table 2). In fact, aerogel impregnation with phenol compounds, which are characterized by a high solubility in ethanol, seems quite effective. By contrast, oil impregnation by mixing with the aqueous phase of the hydrogel is efficacious only if water removal is performed by freeze drying, and thus avoiding possible oil transfer to supercritical CO2 (Comin et al., 2012; Lehtonen et al., 2020). A higher oil loading can be achieved by performing alcogel drying with a mixture of supercritical CO2 and oil (Comin et al., 2012). It has been postulated that the presence of oil in the supercritical CO2 may also assist in the removal of ethanol from the gel pores, although the mechanisms is not clear (Comin et al., 2012).
3.2.2. Post-drying impregnation
In the post-drying impregnation, the active substance is loaded in the dried aerogel. This can be performed by simply immersing the aerogel in the liquid target molecule (Table 2). Such procedure was used for loading aerogels with an anti-fungal volatile (trans-2-hexanal) and can only be applied when the target molecule is a liquid that does not solubilize the aerogel polymer. In most cases, the target molecule to be loaded in the aerogel is generally solubilised in an assisting solvent, which is then allowed to diffuse into the aerogel pores. The subsequent removal of the solvent from the aerogel causes solute precipitation/absorption into the matrix pores. Depending on the characteristic of the target molecule, adequate solvents are selected. For instance, Ahmadi, Madadlou, and Saboury (2016) performed post drying oil impregnation by soaking a starch aerogel in a solution of hexane-oil. Following, hexane was evaporated under hood. Nevertheless, the most efficient and common methodology for post-drying impregnation is currently based on the use of supercritical CO2 as assisting solvent (Table 2). In this case, a supercritical CO2 solution saturated with the target molecule is allowed to diffuse into the aerogel pores. Molecule impregnation would result from chemical adsorption onto the aerogel pores as well as by capillary condensation and local precipitation upon depressurization (Gurikov & Smirnova, 2018). The depressurization of supercritical CO2 is a critical step for impregnation: although fast depressurization is generally associated to higher loadings, slow depressurization allows avoiding the precipitation of the delivered compound onto the surface of the material (Selmer et al., 2019). The latter is certainly undesired when particle agglomeration should be avoided to maintain the typical free-flowing property of dried materials.
Post drying loading assisted by supercritical CO2 has been applied with reference to pharmaceutical compounds (Betz et al., 2012; García-Gonzalez & Smirnova, 2013) as well as food ingredients (Table 2). The solubility of the target compounds in the solvent (i.e. supercritical CO2) is a critical factor controlling the impregnation efficacy (Table 2) (Viganó et al., 2020).
Post drying impregnation of aerogels with non-polar compounds, such as oil, is generally reported to be quite effective, providing loading ratios in the range of 0.2−0.7 g oil/g aerogel (Kleemann et al., 2020; Selmer et al., 2019). By contrast, impregnation of molecules with lower polarity (vitamins or lactulose) seems more critical (Díez-Municio, Montilla, Herrero, Olano, & Ibáñez, 2011; García-González et al., 2015; Ubeyitogullari & Ciftci, 2019). Accordingly, when impregnation involves complex mixtures of molecules with different affinity for SC-CO2, such as oils, the relative abundance of their components in the entrapped oil can be significantly different from that of the original oil. For instance, fish oil entrapped in protein aerogels presented much higher content in triglycerides and cholesterol, and lower content in free fatty acids than fish oil used for loading (Selmer et al., 2019). Comparing data reported in Table 2, it is interesting to note that high loading efficacy of lipids was observed for impregnation into both protein- and polysaccharide-based aerogels. This suggests that the contribution of physical entrapment into the aerogel pores is probably the most critical factor controlling lipid impregnation. In other words, oil absorption into the pores would be driven by number, dimension, interconnectivity and size distribution of pores rather than by the chemical interaction of oil components with the functional groups available on the aerogel surface.
3.2.3. Stability and functionality of aerogel delivery systems
Despite the abundance of papers dealing with impregnated aerogels, limited information is available about their capacity of modifying stability and functionality of the entrapped components. Available data suggest that aerogels would be able to protect sensitive compounds. For instance, entrapping plant extracts into cellulose aerogels was shown to highly maintain their antioxidant activity (De Oliveira et al., 2020). Aerogel coating seems to be critical to decrease oxygen susceptibility of loaded oil. To this regard, Ahmadi et al. (2016) showed that fish oil entrapped in whey protein aerogels coated with zein presented about 60 % lower peroxide value than oil impregnated without coating.
The peculiar physical properties of aerogels are also expected to modify the bioavailability of loaded molecules. To this regard, in vitro bioavailability of phytosterols loaded into starch aerogels resulted significantly higher (35 %) than that of the crude phytosterols (3 %) (Ubeyitogullari, Moreau, Rose, & Ciftci, 2019). The authors also inserted these phytosterol-loaded aerogels into “real” food products, namely granola bars and puddings (Ubeyitogullari & Ciftci, 2019). Introduction of phytosterols in food in the form of aerogel-protected particles made in vitro bioavailability three times higher than when they were added as free ingredients. This effect was attributed to the lower crystallinity level of phytosterols entrapped into the aerogels.
Unlike polysaccharide aerogels which easily dissolve in water, those made of proteins are generally more resistant during swelling and digestion. This is due to the fact that proteins undergo substantial denaturation during hydrogel formation. In addition, hydrogel drying further promotes contraction of the protein backbone, leading to the maximization of the interactions among proteins (Tang, Wei, & Guo, 2014). Due to this water insolubility, the release of loaded molecules is generally delayed (Betz et al., 2012). As an example, fish oil loaded in protein aerogels was mainly released during intestinal digestion, whereas only a small amount was released during oral and gastric digestion (Kleemann et al., 2020).
3.3. Fat replacers
According to their open pore structure and large surface area, aerogels quickly uptake large amounts of oil. This capacity is particularly interesting for the preparation of oleogels, which are mainly proposed as fat substitutes to obtain healthier foods with reduced content of saturated/trans fatty acids (Patel & Dewettinck, 2016; Stortz, Zetzl, Barbut, Cattaruzza, & Marangoni, 2012). According to this application, the oil fraction is driven into the pores of aerogel particles by capillary forces and held at the aerogel surface by surface-oil interactions. For this reason, large amounts of oil closely stick onto the aerogel surface both inside the pores and outside the aerogel particle, which loses the typical dry appearance. The presence of oil at the surface of the hydrophilic particles favors particle-particle interactions, due to intense hydrogen bonding in a nonpolar environment (De Vries, Lopez Gomez, Jansen, van der Linden, & Scholten, 2017). This mechanism allows the formation of a strong particle network, where protein particles behave like building blocks able to embed oil within pores as well as to hold it tightly in the interparticle space (Plazzotta et al., 2020). Absorbed oil is generally higher than 2 g per g of aerogel, regardless of the chemical nature of the aerogel (Table 2). In the case of oleogels obtained from aggregation of whey protein aerogels, the oil content exceeded 5 times the weight of the aerogel particles. The obtained material did not lose any oil upon centrifugation and presented the typical plastic behavior of commercial solid fats (Plazzotta et al., 2020).
4. Applications of aerogels in food packaging
Packaging materials have multiple purposes, being the most important to protect the packed product against mechanical stress, gases and vapors, moisture, light, temperature, microbes, and dirt (Robertson, 2010). Packaging materials are selected based on their capacity to provide this protection, taking into account the other functions that a packaging material may perform, including containing, transporting, serving, presenting the product, and providing information to consumers. Packaging materials can be used as primary packaging, i.e. consumer packaging, or secondary packaging which contains a defined number of primary packages. Secondary packaging units can also be gathered in a tertiary packaging for better transport and storage. The concept of packaging materials also includes a variety of components that can be inserted in the primary packaging to provide further information of the product quality and shelf-life (intelligent packaging), or to extend the shelf-life by adsorbing or releasing functional components (active packaging) (Dobrucka & Przekop, 2019). Other important criteria for packaging materials are their price and environmental impacts, including origin of raw materials, sustainability of processing, and recycling routes. Importantly, consumer experience and user friendliness determine the market potential of packaging materials.
The most important and unique property of aerogels for food packaging is their porous structure, leading to low weight and high specific surface area. This provides interesting opportunities for mechanical protection, thermal insulation, or active packaging materials capable of adsorbing or releasing specific compounds. Aerogel structures also offer an inspiring basis for designers to develop and construct material shapes (Michaloudis & Dann, 2017), which is essential in packaging design.
The mechanical properties of aerogels are determined by their porous morphology (Ghafar et al., 2017). Reinforcing components, such as nanoparticles or fibers can be added to increase the aerogel strength. A practical illustration of the aerogel strength is the weight an aerogel can withstand on it. For example, a chitin-based aerogel square weighing 60 mg and having ∼5.6 cm3 apparent volume withstood an object of 100 g without any shape distortion (Yan et al., 2020). Such strong materials could provide efficient protection to packed food against mechanical stress that could occur during transport or handling. Moisture can alter material properties, especially of materials derived from bio-based polymers. Repeated (minimum five cycles) mechanical milling followed by freezing in liquid nitrogen and thawing partially separated micro- and nanosized fibrils from cellulose fibers, and enabled preparation of dimensionally stable aerogels that retained their shapes and geometric sizes in solutions (Khlebnikov, Postnova, Chen, & Shchipunov, 2020). This is a highly useful property with materials in contact with moist substances, like many foods.
A bio-based thermal insulator, such as aerogel, could be a sustainable replacement for expanded polystyrene used widely to pack products that need either cold storage, such as fish, or maintaining the temperature of hot contents, such as ready-made meals or hot beverages (Mikkonen et al., 2013). Pectin-TiO2 nanocomposite aerogels were proposed for the storage of temperature-sensitive food and prepared via a sol-gel process (Nešić et al., 2018). The thermal conductivity of these aerogels was 0.022–0.025 W/m K, being lower than the thermal conductivity of air (0.024−0.032 W/m K). The thermal conductivity of pectin aerogels followed the aerogel density within a U-shaped curve, where the density depended on preparation conditions, such as cross-linking degree and pH of the solvent (Groult & Budtova, 2018). Pectin aerogels with thermal conductivity as low as 0.0147 ± 0.0002 W/m K were obtained with the optimized preparation method. The conjunction of the thermal insulation and the lightweight is especially attractive for specialized food service conditions like aircraft meal services or crewed spacecraft food, where solutions with reduced fuel consumption is a must.
An active aerogel component was developed to be used to extend the shelf-life of fresh fruit and vegetables (Lehtonen et al., 2020). This innovation was based on in situ production and release of a volatile compound (hexanal), which affects plant metabolism by decreasing ethylene production, and prevents growth of spoilage microbes. The concept was tested with blueberries, where less mold growth was observed, and cherry tomatoes, which maintained their firmness longer when packed with the hexanal-releasing active component in comparison with control samples.
5. Aerogels within EU food regulation
The use of aerogels in foods is not mentioned in current regulation. To this regard, not only the criticisms of production but also the safety issues of the final aerogels require some considerations. Among the advantages of aerogels, literature indicate the absence of hazardous chemicals in the material preparation. Nevertheless, attention should be paid to the use of ethanol in water substitution step. The operation must be performed using ethanol without any denaturant, and considering that volatile residues could remain selectively adsorbed on the high surface area of the aerogel. This might lead to consumption limitations for specific consumers, such as children or people with religious dietary restrictions.
As regards aerogel safety under EU regulation, the question is whether these novel materials can be examined under the current Novel Foods Regulation (EU Regulation 2015/2283, 2015). Based on this regulation, ‘novel food’ means any food that was not used for human consumption within the European Union before 1997, and that falls under a defined list of categories. Among the latter, specific mention is made to food with a new or intentionally modified molecular structure as well as food resulting from technological interventions not previously used. Although not yet used for human consumption, most biopolymer aerogels seem not to fall under this category since they are made with polymers with a long history of safe food use and obtained by drying technologies which have long been used in the food sector.
Nevertheless, in aerogel production, unit operations are combined according to a novel process, in order to intentionally modify the physical structure. The large specific surface area and the pore sizes than come thereof is regarded as a property characteristic of the nanoscale (EU Regulation 2015/2283, 2015). In other words, even if aerogel monoliths or particles have sizes well above the order of 100 nm, their large surface area would account for specific physico-chemical properties that are different from those of the non-nanostructured form of the same polymer. In this sense, aerogels could be considered as engineered nanomaterials.
The debate on aerogels within food regulation is also open to other considerations, including the fact that the surface area of commercial foods obtained by freeze drying and supercritical extraction can fall in the same magnitude range of aerogels. However, in this case, there is a conceptual difference since the large surface area of aerogels is intentionally exploited to functionalise the material and improve its performance. Research on food aerogels is still in its embryonic phase and hence has not yet risen the attention of legislators. More information is certainly needed to distinguish aerogels that can be inserted in the diet from those that will require a specific authorisation.
As regards the use of aerogel as packaging materials, mention should be made to the general principles of safety and inertness for all Food Contact Materials, defined in the Commission Regulation (EC) No 1935/2004. This regulation states that the materials shall not release their constituents into food at levels harmful to human health or that change food composition, taste and odour in an unacceptable way. This should be ensured when aerogels are developed as packaging materials. Active and intelligent materials are considered under specified rules in Commission Regulation (EC) No 450/2009, as by their design they are not inert. Such materials may for example absorb substances from food packaging interior such as liquid and oxygen, release substances into the food such as preservatives, or indicate expiry of food through labelling that changes colour when maximum shelf life or storage temperature is exceeded. Substances permitted for the manufacture of active and intelligent materials are listed in the regulation. New packaging materials, including aerogel delivery systems, must go through safety evaluation as defined in the European Food Safety Authority guidelines (https://efsa.onlinelibrary.wiley.com/doi/epdf/10.2903/j.efsa.2008.21r). One possible solution is to coat the aerogel packaging surface with a barrier layer.
6. Future trends and research needs
An obvious concern with use of aerogels relates to their costs. Bio-based aerogels are typically obtained using highly purified molecules which are costly and produced with considerable waste generation. On the contrary, to allow valorization of industrial discards, which typically represent an environmental and economic burden, aerogels can be prepared according to virtues cycles of circular economy (Budtova et al., 2020). The production of cellulose-based aerogels has been explored using aqueous suspensions of cellulosic fractions of waste biomass, including cane bagasse, lupin hull, corn bracts, rice and oat husks, and spent coffee grounds (Ciftci et al., 2017; De Oliveira et al., 2019, 2020; Fontes-Candia, Erboz, Martinez-Abad, Lopez-Rubio, & Martinez-Sanz, 2019; Jing et al., 2019; Liu, Li, Zhang, Zhu, & Qiu, 2020; Zhang, Kwek, Li, Tan, & Duong, 2019). A further option is based on directly turning cellulose-rich vegetable waste into aerogels with the advantage of simplifying production process (Plazzotta et al., 2018b). Similarly, an increasing number of recent publications explore the possibility to upgrade low-value side streams to obtain aerogels for packaging applications from renewable resources (Alakalhunmaa et al., 2016). Thus the cost contribution of raw materials for aerogel preparation may be low but, on the other hand, a multiple step production process that requires the use of high amounts of solvents, such as supercritical CO2, is costly. Minimization of fresh solvent and continuous CO2 drying process could facilitate aerogel production and reduce costs in industrial scale compared to batch production (Mißfeldt et al., 2020).
Research about aerogel structure in the context of its relationship with molecular composition, processing techniques and potential functionality in food is currently being studied at laboratory level. By contrast, the fate of aerogel ingredients within food materials is almost unknown. Aerogel particles were inserted into two food products, namely, granola bars and puddings, without any specific processing issue, and provided circumstantial evidence that aerogels can be successfully implemented in food formulations (Ubeyitogullari & Ciftci, 2019). Nevertheless, the specific physical properties of aerogels suggest the need for proper adjustments of formulation, processing and storage conditions of aerogel-containing foods. The latter should be then submitted to in vitro studies to clearly highlight their fate in human gut as well as to studies evaluating consumer attitude towards aerogels and market acceptance potentiality.
When considering aerogels for food packaging, two main challenges should be addressed by the aerogel community. Firstly, transparency of bio-based aerogels should be improved. The visual appearance of packaging materials is significant for consumer experience, with transparent materials being often preferred to allow visibility of the packed food. Highly transparent aerogels from oxidized cellulose were recently developed (Plappert et al., 2017) and lead the way to broadening the properties and application range of aerogels. Secondly, after use, packaging materials can be recycled back to material production, burnt for energy, composted, or discarded to landfill. The aerogel composition and its eventual assembling into multicomponent materials will strictly condition the potential recycling options.
Finally, the main issue to be tackled in aerogel technology to become a mainstream solution for food applications is a clear definition of the conditions for their safe use for human consumption and food contact. Testing aerogels with nanostructured properties is not a trivial task, especially when they are inserted in foods, and will require the availability of methods designed to clearly define nature and kinetics of their interaction with biological tissues.
Acknowledgments
Work carried out in the frame of the COST Action CA18125 “Advanced Engineering and Research of aeroGels for Environment and Life Sciences” (AERoGELS) and funded by the European Commission. We thank Dr. Hanna Koivula (University of Helsinki) for fruitful discussions about packaging regulation.
References
Abhari, N., Madadlou, A., & Dini, A. (2017). Structure of starch aerogel as affected by crosslinking and feasibility assessment of the aerogel for an anti-fungal volatile release. Food Chemistry, 221, 147–152. https://doi.org/10.1016/j.foodchem.2016.10.072.
Ahmadi, M., Madadlou, A., & Saboury, A. A. (2016). Whey protein aerogel as blended with cellulose crystalline particles or loaded with fish oil. Food Chemistry, 196,1016–1022.https://doi.org/10.1016/j.foodchem.2015.10.031.
Alakalhunmaa, S., Parikka, K., Penttil¨a, P. A., Cuberes, M. T., Willf¨or, S., Salm´en, L., et al. (2016). Softwood-based sponge gels. Cellulose, 23, 3221–3238. https://doi.org/10.1007/s10570-016-1010-2.
Alnaief, M., Alzaitoun, M. A., García-Gonzalez, C. A., & Smirnova, I. (2011). Preparation of biodegradable nanoporous microspherical aerogel based on alginate. Carbohydrate Polymers, 84, 1011–1018. https://doi.org/10.1016/j.carbpol.2010.12.060.
Antonyuk, S., Heinrich, S., Gurikov, P., Subrahmanyam, R., & Smirnova, I. (2015). Influence of coating and wetting on the mechanical behavior of highly porous cylindrical aerogel particles. Powder Technology, 285, 34–43. https://doi.org/10.1016/j.powtec.2015.05.004.
Arboleda, J. C., Hughes, M., Lucia, L. A., Laine, J., Ekman, K., & Rojas, O. J. (2013). Soy protein-nanocellulose composite aerogels. Cellulose, 20, 2417–2426. https://doi.org/10.1007/s10570-013-9993-4.
Auriemma, G., Russo, P., Del Gaudio, P., García-Gonz´alez, C. A., Landín, M., & Aquino, R. P. (2020). Technologies and formulation design of polysaccharide-based hydrogels for drug delivery. Molecules, 14, 25–29. https://doi.org/10.3390/molecules25143156.
Baudron, V., Gurikov, P., Smirnova, I., & Whitehouse, S. (2019). Porous starch materials via supercritical- and freeze-drying. Gels, 12, 5–25. https://doi.org/10.3390/gels5010012.
Baudron, V., Taboada, M., Gurikov, P., Smirnova, I., & Whitehouse, S. (2020). Production of starch aerogel in form of monoliths and microparticles. Colloid and Polymer Science, 298, 477–494. https://doi.org/10.1007/s00396-020-04616-5.
Betz, M., García-Gonzalez, C. A., Subrahmanyam, R. P., Smirnova, I., & Koluzik, U. (2012). Preparation of novel whey protein-based aerogels as drug carriers for life science. The Journal of Supercritical Fluids, 72, 111–119. https://doi.org/10.1016/j.supflu.2012.08.019.
Bilanovic, D., Starosvetsky, J., & Armon, R. H. (2016). Preparation of biodegradable xanthan-glycerol hydrogel, foam, film, aerogel and xerogel at room temperature. Carbohydrate Polymers, 148, 243–250. https://doi.org/10.1016/j.carbpol.2016.04.058.
Budtova, T. (2019). Cellulose II aerogels: A review. Cellulose, 26, 81–121. https://doi.org/10.1007/s10570-018-2189-1.
Budtova, T., Aguilera, D. A., Beluns, S., Berglund, L., Chartier, C., Espinosa, E., et al. (2020). Biorefinery approach for aerogels. Polymers, 12, 2779. https://doi.org/10.3390/polym12122779.
Bugnone, S., Ronchetti, L., Manna, M., & Banchero, M. (2018). An emulsification/internal setting technique for the preparation of coated and uncoated hybrid silica/alginate aerogel beads for controlled drug delivery. The Journal of Supercritical Fluids,142, 1–9. https://doi.org/10.1016/j.supflu.2018.07.007.
Chen, H.-B., Wang, Y.-Z., & Schiraldi, D. A. (2013). Foam-like materials based on whey protein isolate. European Polymer Journal, 49, 3387–3391. https://doi.org/10.1016/j.eurpolymj.2013.07.019.
Chiu, N., Hewson, L., Yang, N., Linforth, R., & Fisk, I. (2015). Controlling salt and aroma perception through the inclusion of air fillers. LWT-Food Science and Technology, 63,65–70. https://doi.org/10.1016/j.lwt.2015.03.098.
Ciftci, D., Ubeyitogullari, A., Razzera Huerta, R., Ciftci, O. N., Flores, R. A., & Salda˜na, M. D. A. (2017). Lupin hull cellulose nanofibers aerogel preparation by supercritical CO2 and freeze-drying. The Journal of Supercritical Fluids, 127, 137–145. https://doi.org/10.1016/j.supflu.2017.04.002.
Co, E. D., & Marangoni, A. G. (2012). Organogels: An alternative edible oil structuring method. Journal of the American Oil Chemists’ Society, 89, 749–780. https://doi.org/10.1007/s11746-012-2049-3.
Comin, L. M., Temelli, F., & Salda˜na, M. D. A. (2012). Barley β-glucan aerogels as a carrier for flax oil via supercritical CO2. Journal of Food Engineering, 111, 625–631. https://doi.org/10.1016/j.foodeng.2012.03.005.
Comin, L. M., Temelli, F., & Salda˜na, M. D. A. (2015). Flax mucilage and barley betaglucan aerogels obtained using supercritical carbon dioxide: Application as flax lignin carriers. Innovative Food Science and Emerging Technologies, 28, 40–46. https://doi.org/10.1016/j.ifset.2015.01.008.
Davidovich-Pinhas, M., Barbut, S., & Marangoni, A. G. (2015). The gelation of oil using ethyl cellulose. Carbohydrate Polymers, 117, 869–878. https://doi.org/10.1016/jcarbpol.2014.10.035.
De Marco, I., & Reverchon, E. (2017). Starch aerogel loaded with poorly water-soluble vitamins through supercritical CO2 adsorption. Chemical Engineering Research & Design, 119, 221–230. https://doi.org/10.1016/j.cherd.2017.01.024.
De Oliveira, J. P., Bruni, G. P., el Halal, S. L. M., Bertoldi, F. C., Dias, A. R. G., & Da Zavareze, E. R. (2019). Cellulose nanocrystals from rice and oat husks and their application in aerogels for food packaging. International Journal of Biological Macromolecules, 124, 175–184. https://doi.org/10.1016/j.ijbiomac.2018.11.205.
De Oliveira, J. P., Bruni, G. P., Fonseca, L. M., Da Silva, F. T., Da Rocha, J. C., & Da Zavareze, E. R. (2020). Characterization of aerogels as bioactive delivery vehicles produced through the valorization of yerba-mate (Illex paraguariensis). Food Hydrocolloids, 107, Article 105931. https://doi.org/10.1016/j.foodhyd.2020.105931.
De Vries, A., Lopez Gomez, Y., Jansen, B., van der Linden, E., & Scholten, E. (2017). Controlling agglomeration of protein aggregates for structure formation in liquid oil: A sticky business. Applied Materials and Interfaces, 9, 10136–10147. https://doi.org/
10.1021/acsami.7b00443.
Del Gaudio, P., Auriemma, G., Mencherini, T., Porta, G. D., Reverchon, E., & Aquino, R. P. (2013). Design of alginate-based aerogel for nonsteroidal antiinflammatory drugs controlled delivery systems using prilling and supercriticalassisted drying. Journal of Pharmaceutical Sciences, 102, 185–194. https://doi.org/
10.1002/jps.23361.
Díez-Municio, M., Montilla, A., Herrero, M., Olano, A., & Ib´a˜nez, E. (2011). Supercritical CO2 impregnation of lactulose on chitosan: A comparison between scaffolds and microspheres form. The Journal of Supercritical Fluids, 57, 73–79. https://doi.org/10.1016/j.supflu.2011.02.001.
Dobrucka, R., & Przekop, R. (2019). New perspectives in active and intelligent food packaging. Journal of Food Processing and Preservation, 43, Article e14194. https://doi.org/10.1111/jfpp.14194.
Dos Santos, P., Vigano, J., Furtado, G. F., Cunha, R. L., Hubinger, M. D., Rezende, C. A., et al. (2020). Production of resveratrol loaded alginate aerogel: Characterization, mathematical modelling, and study of impregnation. The Journal of Supercritical Fluids, 163, Article 104882. https://doi.org/10.1016/j.supflu.2020.104882.
Du, A., Zhou, B., Zhang, Z. H., & Shen, J. (2013). A special material or a new state of matter: A review and reconsideration of the aerogel. Materials, 6, 941–968. https://doi.org/10.3390/ma6030941.
Eleftheriadou, M., Pyrgiotakis, G., & Demokritou, P. (2017). Nanotechnology to the rescue: Using nano-enabled approaches in microbiological food safety and quality. Current Opinion in Biotechnology, 44, 87–93. https://doi.org/10.1016/j.copbio.2016.11.012.
El-Naggar, M. E., Othman, S. I., Allam, A. A., & Morsy, O. M. (2020). Synthesis, drying process and medical application of polysaccharide-based aerogels. International Journal of Biological Macromolecules, 145, 1115–1128. https://doi.org/10.1016/j.ijbiomac.2019.10.037.
Escudero, R. R., Robitzer, M., Di Renzo, F., & Quignard, F. (2009). Alginate aerogels as adsorbents of polar molecules from liquid hydrocarbons: Hexanol as probe molecule. Carbohydrate Polymers, 75, 52–57. https://doi.org/10.1016/j.carbpol.2008.06.008.
European Union Regulation 2004/1935 on Materials and articles intended to come into contact with food of the European Parliament and of the Council of 27 October 2004 (2004).
European Union Regulation 2009/450 on Active and intelligent materials and articles intended to come into contact with food of the European Parliament and of the Council of 29 May 2009 (2009).
European Union Regulation 2015/2283 on Novel foods of the European Parliament and of the Council of 25 November 2015 (2015).
Fontes-Candia, C., Erboz, E., Martinez-Abad, A., Lopez-Rubio, A., & Martinez-Sanz, M. (2019). Superabsorbent food packaging bioactive cellulose-based aerogels from Arundo donax waste biomass. Food Hydrocolloids, 96, 151–160. https://doi.org/10.1016/j.foodhyd.2019.05.011.
Fu, P. P., Xia, Q., Hwang, H.-M., Ray, P. C., & Yu, H. (2014). Mechanisms of nanotoxicity: Generation of reactive oxygen species. Journal of Food and Drug Analysis, 22, 64–75. https://doi.org/10.1016/j.jfda.2014.01.005.
Gaˇcanin, J., Synatschke, C. V., & Weil, T. (2019). Biomedical applications of DNA-based hydrogels. Advanced Functional Materials, 30, Article 1906253. https://doi.org/10.1002/adfm.201906253.
Ganesan, K., Budtova, T., Ratke, L., Gurikov, P., Baudron, V., Preibisch, I., et al. (2018). Review on the production of polysaccharide aerogel particles. Materials, 2144,11–28. https://doi.org/10.3390/ma11112144.
Gao, R., Lu, Y., Xiao, S., & Li, J. (2017). Facile fabrication of nanofibrillated chitin/Ag(2) O heterostructured aerogels with high iodine capture efficiency. Scientific Reports, 7,4303. https://doi.org/10.1038/s41598-017-04436-8.
García-Gonzalez, C. A., & Smirnova, I. (2013). Use of supercritical fluid technology for the production of tailor-made aerogel particles for delivery systems. The Journal of Supercritical Fluids, 79, 152–158. https://doi.org/10.1016/j.supflu.2013.03.001.
García-Gonzalez, C. A., Alnaief, M., & Smirnova, I. (2011). Polysaccharide-based aerogels – Promising biodegradable carriers for frug delivery systems. Carbohydrate Polymers, 86, 1426–1438. https://doi.org/10.1016/j.carbpol.2011.06.066. García-Gonz´alez, C. A., Budtova, T., Dur˜aes, L., Erkey, C., Del Gaudio, P., Gurikov, P.,
et al. (2019). An opinion paper on aerogels for biomedical and environmental applications. Molecules, 24, 15. https://doi.org/10.3390/molecules24091815.
García-Gonz´alez, C. A., Jin, M., Gerth, J., Alvarez-Lorenzo, C., & Smirnova, I. (2015). Polysaccharide-based aerogel microspheres for oral drug delivery. Carbohydrate Polymers, 117, 797–806. https://doi.org/10.1016/j.carbpol.2014.10.045.
García-Gonz´alez, C. A., Sosnik, A., Kalmar, J., De Marco, I., Erkey, C., Concheiro, A., et al. (2021). Aerogels in drug delivery: From design to application. Journal of Controlled Release, 332, 40–63. https://doi.org/10.1016/j.jconrel.2021.02.012.
Gesser, H. D., & Goswami, P. C. (1989). Aerogels and related porous materials. Chemical Reviews, 89, 765–788. https://doi.org/10.1021/cr00094a003.
Ghafar, A., Parikka, K., Haberthür, D., Tenkanen, M., Mikkonen, K. S., & Suuronen, J.-P. (2017). Synchrotron microtomography reveals the fine three-dimensional porosity of composite polysaccharide aerogels. Materials, 10, 871.
Ghanbarzadeh, B., Oleyaei, S. A., & Almasi, H. (2015). Nanostructured materials utilized in biopolymer-based plastics for food packaging applications. Critical Reviews in Food Science and Nutrition, 55(12), 1699–1723. https://doi.org/10.1080/10408398.2012.731023.
Goh, S. M., Leroux, B., Groeneschild, C. A. G., & Busch, J. (2010). On the effect of tastant excluded fillers on sweetness and saltiness of a model food. Journal of Food Science, 75(4), 245–249. https://doi.org/10.1111/j.1750-3841.2010.01597.x.
Groult, S., & Budtova, T. (2018). Thermal conductivity/structure correlations in thermal super insulating pectin aerogels. Carbohydrate Polymers, 196, 73–81. https://doi.org/10.1016/j.carbpol.2018.05.026.
Gurikov, P., & Smirnova, I. (2018). Amorphization of drugs by adsorptive precipitation from supercritical solutions: A review. The Journal of Supercritical Fluids, 132, 105–125. https://doi.org/10.1016/j.supflu.2017.03.005.
Haimer, E., Wendland, M., Schlufter, K., Frankenfeld, K., Miethe, P., Potthast, A., et al. (2010). Loading of bacterial cellulose aerogels with bioactive compounds by antisolvent precipitation with supercritical carbon dioxide. Macromolecular Symposia, 294, 64–74. https://doi.org/10.1016/j.foodhyd.2017.04.021.
Huang, Y., He, M., Lu, A., Zhou, W. Z., Stoyanov, S. D., Pelan, E. G., et al. (2015). Hydrophobic modification of chitin whiskers and its potential application in structuring oil. Langmuir, 31, 1641–1648. https://doi.org/10.1021/la504576p.
Ivanovic, J., Milovanovic, S., & Zizovic, I. (2016). Utilization of supercritical CO2 as a processing aid in setting functionality of starch-based materials. Starch/St¨arke, 68,821–833. https://doi.org/10.1002/star.201500194.
Jim´enez-Saelices, C., Seantier, B., Cathala, B., & Grohens, Y. (2017). Spray freeze-dried nanofibrillated cellulose aerogels with thermal superinsulating properties. Carbohydrate Polymers, 157, 105–113. https://doi.org/10.1016/j.carbpol.2016.09.068.
Jing, F., Ding, J., Zhang, T., Yang, D., Qiu, F., Chen, Q., et al. (2019). Flexible, versatility and superhydrophobic biomass carbon aerogels derived from corn bracts for efficient oil/water separation. Food and Bioproducts Processing, 115, 134–142. https://doi.org/10.1016/J.FBP.2019.03.010.
Khlebnikov, O. N., Postnova, I. V., Chen, L.-J., & Shchipunov, Y. A. (2020). Silication of dimensionally stable cellulose aerogels for improving their mechanical properties.Colloid Journal, 82, 448–459. https://doi.org/10.1134/S1061933X20040043.
Kistler, S. S. (1931). Coherent expanded aerogels and jellies. Nature, 127(3211), 741. https://doi.org/10.1038/127741a0.
Kistler, S. S. (1932). Coherent expanded aerogels. Journal of Physical Chemistry, 36,52–60. https://doi.org/10.1021/j150331a003.
Kleemann, C., Schusater, R., Rosenecker, E., Selmer, I., Smirnova, I., & Kulozik, U. (2020). In-vitro digestion and swelling kinetics of whey protein, egg white protein and sodium caseinate aerogels. Food Hydrocolloids, 101, Article 105534. https://doi.org/10.1016/j.foodhyd.2019.105534.
Kleemann, C., Selmer, I., Smirnova, I., & Kulozik, U. (2018). Tailor made protein based aerogel particles from egg white protein, whey protein isolate and sodium casinate: Influence of the preceding hydrogel characteristics. Food Hydrocolloids, 83, 365–374. https://doi.org/10.1016/j.foodhyd.2018.05.021.
Laredo, T., Barbut, S., & Marangoni, A. G. (2011). Molecular interactions of polymer oleogelation. Soft Matter, 6, 2734–2743. https://doi.org/10.1039/c0sm00885k.
Lehtonen, M., Kek¨al¨ainen, S., Nikkil¨a, I., Kilpel¨ainen, P., Tenkanen, M., & Mikkonen, K. S. (2020). Active food packaging through controlled in situ production and release of hexanal. Food Chemistry: X, 5, Article 100074, 10.1016/j.foodhyd.2018.05.021.
Li, Y., Grishkewich, N., Liu, L., Wang, C., Tam, K. C., Liu, S., et al. (2019). Construction of functional cellulose aerogels via atmospheric drying chemically cross-linked and solvent exchanged cellulose nanofibrils. Chemical Engineering Journal, 366, 531–538. https://doi.org/10.1016/j.cej.2019.02.111.
Li, Z. L., Ge, Y. Y., & Wan, L. (2015). Fabrication of a green porous lignin-based sphere for the removal of lead ions from aqueous media. Journal of Hazardous Materials, 285, 77–83. https://doi.org/10.1016/j.jhazmat.2014.11.033.
Liu, H., Li, P., Zhang, T., Zhu, Y., & Qiu, F. (2020). Fabrication of recyclable magnetic double-base aerogel with waste bioresource bagasse as the source of fiber for the enhanced removal of chromium ions from aqueous solution. Food and Bioproducts Processing, 119, 257–267. https://doi.org/10.1016/J.FBP.2019.11.010.
Liu, S. K., Zhou, C. C., Mou, S., Li, J. L., Zhou, Zeng, Y. Y., et al. (2019). Biocompatible graphene oxide-collagen composite aerogel for enhanced stiffness and in situ bone regeneration. Material Science & Engineering C-Materials for Biological Applications, 105, Article 110137. https://doi.org/10.1016/j.msec.2019.110137.
Mallepally, R. R., Bernard, I., Marin, M. A., Ward, K. R., & McHugh, M. A. (2013). Superabsorbent alginate aerogels. The Journal of Supercritical Fluids, 79, 202–208.
Manzocco, L., Basso, F., Plazzotta, S., & Calligaris, S. (2021). Study on the possibility of developing food-grade hydrophobic bio-aerogels by using an oleogel template approach. Current Research in Food Science. https://doi.org/10.1016/j.crfs.2021.02.005. in press, Available on line as Journal pre-proof, 20 Februrary2021.
Manzocco, L., Valoppi, F., Calligaris, S., Andreatta, F., Spilimbergo, S., & Nicoli, M. C. (2017). Exploitation of k-carrageenan aerogels as template for edible oleogel preparation. Food Hydrocolloids, 71, 68–75. https://doi.org/10.1016/j.foodhyd.2017.04.021.
Mehling, T., Smirnova, I., Guenther, U., & Neubert, R. H. H. (2009). Polysaccharidebased aerogels as drug carriers. Journal of Non- Crystalline Solids, 355, 2472–2479. https://doi.org/10.1016/j.jnoncrysol.2009.08.038.
Michaloudis, I., & Dann, B. (2017). Aer( )sculpture: Inventing skies and micro-clouds into diaphanous sculptures made of the space technology nanomaterial silica aerogel. Journal pf Sol-Gel Science and Technology, 84, 535–542. https://doi.org/10.1007/s10971-017-4370-7.
Miguel, F., Martín, A., Gamse, T., & Cocero, M. J. (2006). Supercritical anti solvent precipitation of lycopene: Effect of the operating parameters. The Journal of Supercritical Fluids, 36, 225–235. https://doi.org/10.1016/j.supflu.2005.06.009.
Mikkonen, K. S., Parikka, K., Ghafar, A., & Tenkanen, M. (2013). Prospects of polysaccharide aerogels as modern advanced food materials. Trends in Food Science & Technology, 34, 124–136. https://doi.org/10.1016/j.tifs.2013.10.003.
Mikkonen, K. S., Parikka, K., Suuronen, J.-P., Ghafar, A., Serimaa, R., & Tenkanen, M. (2014). Enzymatic oxidation as a potential new route to produce polysaccharide aerogels. RSC Advances, 4, 11884–11892. https://doi.org/10.1039/c3ra47440b.
Miranda-Tavares, G., Croguennec, T., Carvalho, A. F., & Bouhallab, S. (2014). Milk proteins as encapsulation devices and delivery vehicles: Applications and trends. Trends in Food Science and Technology, 37, 5–20. https://doi.org/10.1016/j.tifs.2014.02.008.
Mißfeldt, F., Gurikov, P., L¨olsberg, W., Weinrich, D., Lied, F., Fricke, M., et al. (2020). Continuous supercritical drying of aerogel particles: Proof of concept. Industrial & Engineering Chemistry Research., 59(24), 11284–11295. https://doi.org/10.1021/acs.iecr.0c01356.
Munoz-Ruiz, A., Escobar-García, D. M., Quintana, M., Pozos-Guillen, A., Pozos-Guillen, A., & Flores, H. (2019). Synthesis and characterization of a new collagenalginate aerogel for tissue engineering. Journal of Nanomaterials, 2019, Article 2875375. https://doi.org/10.1155/2019/2875375.
Nemoto, J., Saito, T., & Isogai, A. (2015). Simple freeze-drying procedure for producing nanocellulose aerogel-containing, high-performance air filters. ACS Applied Materials & Interfaces, 7, 19809–19815. https://doi.org/10.1021/acsami.5b05841.
Neˇsi´c, A., Gordic, M., Davidovi´c, S., Radovanovi´c, ˇZ., Nedeljkovi´c, J., Smirnova, I., et al.(2018). Pectin-based nanocomposite aerogels for potential insulated food packaging application. Carbohydrate Polymers, 195, 128–135. https://doi.org/10.1016/j.carbpol.2018.04.076.
Nikiforidis, C. V., & Scholten, E. (2015). Polymer organogelation with chitin and chitin nanocrystals. RSC Advances, 5, 37789–37799. https://doi.org/10.1039/c5ra06451a.
Nita, L. E., Ghilan, A., Rusu, A. G., Neamtu, I., & Chiriac, A. P. (2020). New trends in biobased aerogels. Pharmaceutics, 12, 449. https://doi.org/10.3390/pharmaceutics12050449.
Osterholt, K. M., Liane, L., Roe, S., & Rolls, B. J. (2007). Incorporation of air into a snack food reduces energy intake. Appetite, 3, 351–358. https://doi.org/10.1016/j.appet.2006.10.007.
Panti´c, M., Knez, ˇZ., & Novak, Z. (2016). Supercritical impregnation as a feasible technique for entrapment of fat-soluble vitamins into alginate aerogels. Journal of Non- Crystalline Solids, 432, 519–526. https://doi.org/10.1016/j.jnoncrysol.2015.11.011.
Panti´c, M., Kotnik, P., Knez, ˇZ., & Novak, Z. (2016). High pressure impregnation of vitamin D3 into polysaccharides aerogels using moderate and low temperatures. The Journal of Supercritical Fluids, 118, 171–177. https://doi.org/10.1016/j.supflu.2016.08.008.
Parikka, K., Nikkila, I., Pitkanen, L., Ghafar, A., Sontag-Strohm, T., & Tenkanen, M. (2017). Laccase/TEMPO oxidation in the production of mechanically strong arabinoxylan and glucomannan aerogels. Carbohydrate Polymers, 175, 377–386. https://doi.org/10.1016/j.carbpol.2017.07.074.
Patel, A. R. (2018). Structuring edible oils with hydrocolloids: where do we stand? Food Biophysics, 13, 113–115. https://doi.org/10.1007/s11483-018-9527-6.
Patel, A. R., & Dewettinck, K. (2016). Edible oil structuring: An overview and recent updates. Food & Function, 7, 20–29. https://doi.org/10.1039/c5fo01006c.
Pathakoti, K., Manubolu, M., & Hwang, H.-M. (2017). Nanostructures: Current uses and future applications in food science. Journal of Food and Drug Analysis, 25, 245–253.
https://doi.org/10.1016/j.jfda.2017.02.004.
Pierre, A. C., & Pajonk, G. M. (2002). Chemistry of aerogels and their applications. Chemical Reviews, 102, 4243–4265. https://doi.org/10.1021/cr0101306.
Plappert, S. F., Nedelec, J.-M., Rennhofer, H., Lichtenegger, H. C., & Liebner, F. W. (2017). Strain hardening and pore size harmonization by uniaxial densification: A facile approach toward superinsulating aerogels from nematic nanofibrillated 2,3-dicarboxyl cellulose. Chemistry of Materials, 29(16), 6630–166641. https://doi.org/10.1021/acs.chemmater.7b00787.
Plazzotta, S., Calligaris, S., & Manzocco, L. (2020). Structural characterisation of oleogels from whey protein aerogel particles. Food Research International, 132, Article 109099, 10.1016/j.foodres.2020.109099.
Plazzotta, S., Calligaris, S., & Manzocco, L. (2018a). Application of different drying techniques to fresh-cut salad waste to obtain food ingredients rich in antioxidants and with high solvent loading capacity. LWT- Food Science and Technology, 89, 276–283. https://doi.org/10.1016/j.lwt.2017.10.056.
Plazzotta, S., Calligaris, S., & Manzocco, L. (2018b). Innovative bioaerogel materials from fresh-cut salad waste via supercritical-CO2-drying. Innovative Food Science and Emerging Technologies, 47, 485–492. https://doi.org/10.1016/j.ifset.2018.04.022.
Robertson, G. L. (2010). Food packaging and shelf life. Food packaging and shelf life – A practical guide (pp. 1–16). Boca Raton, FL: CRC Press, Taylor & Francis Group.
Rodríguez-Dorado, R., L´opez-Iglesias, C., García-Gonz´alez, C. A., Auriemma, G., Aquino, R. P., & Del Gaudio, P. (2019). Design of aerogels, cryogels and xerogels of alginate: Effect of molecular weight, gelation conditions and drying method on
particles’ micromeritics. Molecules, 24, 1049. https://doi.org/10.3390/molecules24061049.
Romoscanu, & Mezzenga. (2006). Emulsion-templated fully reversible protein-in-oil gels. Langmuir, 22, 7812–7818. https://doi.org/10.1021/la060878p.
S¸ahin, ˙I., ¨Ozbakır, Y., ˙In¨onü, Z., Ulker, Z., & Erkey, C. (2017). Kinetics of supercritical drying of gels. Gels, 4, 4–27. https://doi.org/10.3390/gels4010003.
Santos-Rosales, V., Ardao, I., Alvarez-Lorenzo, C., Ribeiro, N., Oliveira, A. L., & García-Gonzalez, C. A. (2019). Sterile and dual-porous aerogels scaffolds obtained through a multistep supercritical CO2-based approach. Molecules, 24, 871. https://doi.org/10.3390/molecules24050871.
Selmer, I., Karnetzke, J., Kleemann, C., Lehtonen, M., Mikkonen, K. S., Kulozik, U., et al.(2019). Encapsulation of fish oil in protein aerogel micro-particles. Journal of Food Engineering, 260, 1–11. https://doi.org/10.1016/j.jfoodeng.2019.04.016.
Selmer, I., Kleemann, C., Kulozik, U., Heinrich, S., & Smirnova, I. (2015). Development of egg white protein aerogels as new material for microencapsulation in food. The Journal of Supercritical Fluids, 106, 42–49. https://doi.org/10.1016/j.supflu.2015.05.023.
Stortz, T. A., Zetzl, A. K., Barbut, S., Cattaruzza, A., & Marangoni, A. G. (2012). Edible oleogels in food products to help maximize health benefits and improve nutritional profiles. Lipid Technology, 24(7), 151. https://doi.org/10.1002/lite.201200205.
Tang, Z., Wei, Q., & Guo, B. (2014). A generic solvent exchange method to disperse MoS2 in organic solvents to ease the solution process. Chemical Communications, 50, 3934–3937. https://doi.org/10.1039/c4cc00425f.
T´erech, P., & Weiss, R. G. (1997). Low molecular mass gelators of
organic liquids and the properties of their gels. Chemical Reviews, 97, 3133–3159. https://doi.org/10.1021/cr9700282.
Tkalec, G., Knez, ˇZ, & Novak, Z. (2016). PH sensitive mesoporous materials for immediate or controlled release of NSAID. Microporous and Mesoporous Materials, 224, 190–200. https://doi.org/10.1016/j.micromeso.2015.11.048.
Ubeyitogullari, A., & Ciftci, O. N. (2016). Formation of nanoporous
aerogels from wheat starch. Carbohydrate Polymers, 147, 125–132. https://doi.org/10.1016/j.carbpol.2016.03.086.
Ubeyitogullari, A., & Ciftci, O. N. (2019). In vitro bioaccessibility of novel lowcrystallinity phytosterol nanoparticles in non-fat and regular-fat foods. Food Research International, 123, 27–35. https://doi.org/10.1016/j.foodres.2019.04.014.
Ubeyitogullari, A., & Ciftci, O. N. (2020). Fabrication of bioaerogels from camelina seed mucilage for food applications. Food Hydrocolloids, 102, Article 105597. https://doi.org/10.1016/j.foodhyd.2019.105597.
Ubeyitogullari, A., Brahma, S., Rose, D. J., & Ciftci, O. N. (2018). In vitro digestibility of nanoporous wheat starch aerogels. Journal of Agricultural and Food Chemistry, 66, 9490–9497. https://doi.org/10.1021/acs.jafc.8b03231.
Ubeyitogullari, A., Moreau, R., Rose, D. J., & Ciftci, O. N. (2019). In vitro bioaccessibility of low-crystallinity phytosterol nanoparticles generated using nanoporous starch bioaerogels. Journal of Food Science, 84(7), 1812–1919. https://doi.org/10.1111/1750-3841.14673.
Veronovski, A., Knez, ˇZ, & Novak, Z. (2013). Comparison of ionic and non-ionic drug release from multi-membrane spherical aerogels. International Journal of Pharmaceutics, 454, 58–66. https://doi.org/10.1016/j.ijpharm.2013.06.074.
Vigan´o, J., Meirelles, A. A. D., Nathia-Neves, G., Baseggio, A. M., Cunha, R. L., Junior, M. R. M., et al. (2020). Impregnation of passion fruit bagasse extract in alginate aerogel microparticles. International Journal of Biological Macromolecules, 155, 1060–1068. https://doi.org/10.1016/j.ijbiomac.2019.11.070.
White, R. J., Budarin, V. L., & Clark, J. H. (2010). Pectin-derived porous materials. Chemistry European Journal, 16, 1326–1335. https://doi.org/10.1002/chem.200901879.
Yan, Y., Ge, F., Qin, Y., Ruan, M., Guo, Z., He, C., et al. (2020). Ultralight and robust aerogels based on nanochitin towards water-resistant thermal insulators. Carbohydrate Polymers, 248, Article 116755. https://doi.org/10.1016/j.carbpol.2020.116755.
Zeynep, U., & Erkey, C. (2014). An emerging platform for drug delivery: Aerogel based systems. Journal of Controlled Release, 177, 51–63. https://doi.org/10.1016/j.jconrel.2013.12.033.
Zhang, X., Kwek, L. P., Li, D. K., Tan, M. S., & Duong, H. M. (2019). Fabrication and properties of hybrid coffee-cellulose aerogels from spent coffee grounds. Polymers, 11, 1942. https://doi.org/10.3390/polym11121942.
Zhao, S., Malfait, W. J., Guerrero-Alburquerque, N., Koebel, M. M., & Nystr¨om, G. (2018). Biopolymer aerogels and foams: Chemistry, properties, and applications. Angewandte Chemie, 57, 7580–7608. https://doi.org/10.1002/anie.201709014.
Zheng, Q., Tian, Y., Ye, F., Zhou, Y., & Zhao, G. (2020). Fabrication and application of starch-based aerogels: Technical strategies. Trends in Food Science & Technology, 99, 608–620. https://doi.org/10.1016/j.tifs.2020.03.038.